Documentation Revision Date: 2025-03-25
Dataset Version: 1
Summary
There are 7,671 data files in NetCDF version 4 format (.nc4) with this dataset: one file for each day of the year for the years 2000 through 2020.
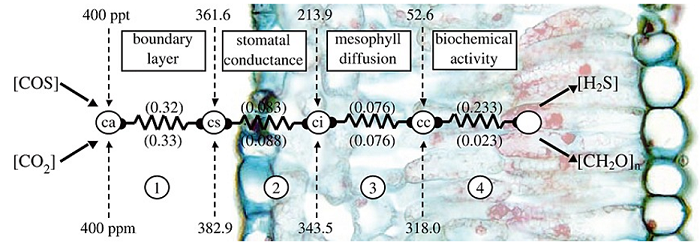
Figure 1. SiB4 COS Uptake-Resistance Model. Numbers in parentheses are conductance values corresponding to the processes in the square boxes: (1) Boundary layer conductance, (2) Stomatal conductance, (3) Mesophyll conductance, and (4) Biochemical rate constant. For COS, we take steps 3 and 4 together to produce an apparent COS mesophyll conductance (variable cosgm in dataset). This figure was taken from Berry et al. (2013).
Citation
Kaushik, A., L. Hu, and J.B. Miller. 2024. SiB4 Modeled 0.5-degree Carbonyl Sulfide Vegetation and Soil Fluxes, 2000-2020. ORNL DAAC, Oak Ridge, Tennessee, USA. https://doi.org/10.3334/ORNLDAAC/2324
Table of Contents
- Dataset Overview
- Data Characteristics
- Application and Derivation
- Quality Assessment
- Data Acquisition, Materials, and Methods
- Data Access
- References
Dataset Overview
This dataset provides outputs from the Simple Biosphere Model (v 4.2) using a modified methodology from Haynes et al. (2021). Products include hourly 0.5-degree gridded fluxes of gross primary productivity (GPP), respiration, carbonyl sulfide (COS) uptake by vegetation and soil, along with conductance of COS (apparent mesophyll and total), stomatal conductance of water and partial pressure of CO2 in the canopy air space, leaf surface, interior and chloroplast. The data are separated by plant functional type (PFT). Fluxes have dimensions of latitude, longitude, time, and plant functional type. Model output spans 53N to 90N latitude and 180W to 180E longitude over years 2000 to 2020.
Project: ABoVE
The Arctic-Boreal Vulnerability Experiment (ABoVE) is a NASA Terrestrial Ecology Program field campaign being conducted in Alaska and western Canada, for 8 to 10 years, starting in 2015. Research for ABoVE links field-based, process-level studies with geospatial data products derived from airborne and satellite sensors, providing a foundation for improving the analysis, and modeling capabilities needed to understand and predict ecosystem responses to, and societal implications of, climate change in the Arctic and Boreal regions.
Related Dataset
Haynes, K.D., I.T. Baker, and A.S. Denning. 2021. SiB4 Modeled Global 0.5-Degree Monthly Carbon Fluxes and Pools, 2000-2018. ORNL DAAC, Oak Ridge, Tennessee, USA. https://doi.org/10.3334/ORNLDAAC/1848
- The methodology employed was taken from this dataset with modifications: After performing an equilibrium spin-up, a varying CO2 boundary condition was employed during the simulation period. This was implemented using NOAA data to distribute monthly mean CO2 values across a global grid as part of the forcing data.
Acknowledgments
This study was funded by the NASA ABoVE Program (grant 80NSSC19M0105). SiB4 development was also supported in part by funding from the NOAA Climate Program Office Atmospheric Chemistry Carbon Cycle and Climate program.
Data Characteristics
Spatial Coverage: Circumpolar, latitudes > 53 degrees north
Spatial Resolution: 0.5 degree
Temporal Coverage: 2000-01-01 to 2020-12-31
Temporal Resolution: Daily files with hourly data
Study Area: Latitude and longitude are given in decimal degrees.
Site | Westernmost Longitude | Easternmost Longitude | Northernmost Latitude | Southernmost Latitude |
---|---|---|---|---|
Pan-arctic | -180 | 180 | 90 | 53 |
Data File Information
There are 7,671 data files in NetCDF version 4 format (.nc4) with this dataset: one file for each day of the year for the years 2000 through 2020. Each data file contains both modeled variables and input variables, as described in Tables 1 and 2. Plant functional types (PFT) are listed in Table 3.
The files are named sib4-hourly-<YYYY-MM-DD>.nc4, where <YYYY-MM-DD> is each day of all months for the period 2000-01-01 to 2020-12-31.
Example file name: sib4-hourly-2000-01-26.nc4
Table 1. Modeled variables.
Variable | Dimensions | Units | Description |
---|---|---|---|
assim | lon, lat, npft, time | µmole m-2 s-1 | Assimilation (or Gross Primary Productivity) |
cos_assim | lon, lat, npft, time | pmole m-2 s-1 | Carbonyl Sulfide (COS) Vegetation Assimilation |
cos_grnd | lon, lat, npft, time | pmole m-2 s-1 | COS Soil Uptake |
cosgm | lon, lat, npft, time | mole m-2 s-1 | Apparent mesophyll conductance of COS |
cosgt | lon, lat, npft, time | mole m-2 s-1 | Total conductance of COS |
gsh2o | lon, lat, npft, time | mole m-2 s-1 | Stomatal conductance of water (H2O) |
resp_auto | lon, lat, npft, time | µmole m-2 s-1 | Autotrophic Respiration |
resp_het | lon, lat, npft, time | µmole m-2 s-1 | Heterotrophic Respiration |
pco2cas | lon, lat, npft, time | Pa | Canopy Air Space CO2 partial pressure |
pco2s | lon, lat, npft, time | Pa | Leaf Surface CO2 partial pressure |
pco2i | lon, lat, npft, time | Pa | Leaf Internal CO2 partial pressure |
pco2c | lon, lat, npft, time | Pa | Leaf Chloroplast CO2 partial pressure |
Table 2. Input variable descriptions.
Variable | Dimensions | Units | Description |
---|---|---|---|
crs | - | - | The Coordinate Reference System, WGS84 (EPSG:4326) |
lat | lat | degrees north | Latitude |
lon | lon | degrees east | Longitude |
pft_area | lon, lat, npft | 1 | Fractional areal coverage of PFT in each grid cell |
pft_names | npft, clen | - | Names of 15 plant functional types (PFT); three-character string abbreviations (clen) defined in Table 3. Output variables are partitioned by PFT and indexed by the npft dimension. |
time | time | d | Timestep in hours since the beginning of 2000-01-01 |
time_bnds | time, bnds | d | Timestep in hours since the beginning of 2000-01-01 (bnds=2) |
Table 3. Plant functional types (PFT) from Haynes et al. (2020). npft and clen are dimensions of variable pft_names (Table 2).
npft value | clen value | Plant Functional Type |
---|---|---|
1 | DBG | Desert and Bare Ground |
2 | ENF | Evergreen Needleleaf Forest |
3 | DNF | Deciduous Needleleaf Forest |
4 | EBF | Evergreen Broadleaf Forest |
5 | DBF | Deciduous Broadleaf Forest |
6 | SHB | Shrubs (Non-Tundra) |
7 | SHA | Tundra Shrubs |
8 | C3A | Tundra Grassland |
9 | C3G | C3 Grassland |
10 | C4G | C4 Grassland |
11 | C3C | C3 Generic Crop |
12 | C4C | C4 Generic Crop |
13 | MZE | Maize |
14 | SOY | Soybeans |
15 | WWT | Winter Wheat |
Application and Derivation
CO2 fluxes are used to study terrestrial ecosystem changes in response to climate warming. COS fluxes are used in the carbon cycle community as a proxy for gross plant uptake and for plant stomatal conductance. The output variables were used for calculating the seasonal cycle trends and results explored in the submitted manuscript.
Quality Assessment
The model was run once with a fixed set of parameters. This study does not include uncertainty based on parameter selection. Annual GPP values included in this dataset were found to be reasonable compared to other datasets when tested with the ILAMB framework (unpublished). Previous SiB4 datasets published on the ORNL DAAC have included more extensive comparisons with other datasets (e.g. see QA section in the ORNL DAAC related dataset https://doi.org/10.3334/ORNLDAAC/1848).
Data Acquisition, Materials, and Methods
This dataset is output from a Simple Biosphere Model (SiB4) simulation, following SiB4 methodology described in Haynes et al. (2019, 2021). SiB4 OCS simulations are described in Section 7 of the SiB4 Tech note (Haynes et al., 2020), and the methodology is summarized below. CO2 simulations were performed following the methodology described in Haynes et al. (2021). The only modification is that after performing an equilibrium spin-up, a varying CO2 boundary condition was employed during the simulation period. This was implemented using NOAA data to distribute monthly mean CO2 values across a global grid as part of the forcing data.
Carbonyl sulfide (COS) is an atmospheric trace gas analog of carbon dioxide (CO2). While CO2 is both taken up and emitted during interactions with plants (through photosynthesis and respiration, respectively), COS is irreversibly hydrolyzed inside the leaf once it is taken up. In recent years, COS has been used as a proxy for plant carbon uptake. While there are other sources and sinks that potentially complicate this relationship, they are generally small compared to the magnitude of plant uptake (Whelan et al., 2018). COS has thus been used as a tracer for gross plant uptake and stomatal conductance at site and regional scales (e.g., Seibt et al. (2010), Montzka et al. (2007), Wehr et al. (2017), Hilton et al. (2017), Hu et al. (2021), Kooijmans et al. (2021)).
The implementation of COS plant uptake in SiB4 is based on the mechanistic and empirical descriptions of leaf uptake described in Berry et al. (2013). Atmospheric COS is taken up by plants and consumed inside leaf chloroplasts by the enzyme carbonic anhydrase (CA). Since COS takes the same pathway as CO2, the series of resistances is similar and this is illustrated in Figure 1.
Relative to water (H2O), the greater mass and larger cross-section of COS results in diffusion restriction by a factor of 1.94 in the stomata and 1.56 in the laminar boundary layer. Once inside the leaf cell, COS hydrolysis is catalyzed by CA at a rate proportional to the internal partial pressure of COS. The combined mesophyll conductance and biochemical CA activity (‘apparent mesophyll conductance’, or gmcos) scales with photosynthetic capacity Vmax such that: gmcos = α * Vmax, where α is a parameter that is calibrated to observations (Stimler et al., 2012). Using this simplified framework, COS uptake by plants (assimcos) is written as:
,
where COSCAS is the canopy air space COS mole fraction, gtcos is calculated from the respective water vapor conductances to boundary layer (gbh2o) and stomata (gsh2o), C4 is a one or zero respectively depending on whether or not the plant is C4 or C3. APARkk is the scaling factor for leaf radiation, RSTFAC2 is the root zone water potential, P is the pressure, P0sfc is the reference surface pressure (10,000 Pa), Tcan is the canopy temperature, and Tice is the freezing temperature of water (273.15 K).
In addition to plant uptake, COS is also taken up by soils. Soil COS exchange is modeled in SiB4 following the mechanistic soil model described in Kooijmans et al. (2021). Briefly, field and laboratory experiments have shown that soil both takes up and emits COS, and that emission due to abiotic thermal degradation and photodegradation of soil organic matter can be particularly pronounced in agricultural soils (Meredith et al., 2018). A new mechanistic model describes the uptake and production pathways together in the soil column (Ogée et al., 2016). Due to recent efforts, parameter values are now available for a range of biomes and land cover types. The COS soil flux calculation is as follows:
,
where k is the CA reaction rate (s-1), B is the solubility of COS in water (cubic meters of water per cubic meter of air), θ is the soil water content (m3 m-3), D is the soil COS diffusivity (cubic meters of air per meter of soil per second), Ca is the canopy air space COS mole fraction, z12 is D / (kBθ), and P is the uniform COS production rate over depth zp (here assumed to be 1.0 m). Soil COS diffusivity further depends on (a) soil porosity (calculated from sand fractions following Lawrence and Slater (2008)), (b) the layer used for soil temperature and soil moisture (taken from the top 5-cm layer, where most of the OCS uptake and emissions are assumed to take place), and (c) tortuosity functions (using Deepagoda et al. (2011) for air and Millington and Quirk (1961) for water). Uptake through hydrolysis in soil water depends on soil CA enzyme activity. Following Meredith et al. (2019), this is expressed as:
,
where fCA is the CA enhancement factor, kuncat varies with soil pH following Elliott et al. (1989), and xCA(T) and xCA(Tref) are temperature response functions from Ogée et al. (2016). Biome-averaged fCA from Meredith et al. (2019) was used to calculate COS uptake for different PFTs.
COS production (Pcos) is a temperature-dependent response function modulated by soil redox potential. Following Meredith et al. (2018), this exponential temperature model was used with biome-averaged values for constants a and b.
The COS parameters used in the calculations described are listed in Figure 2.
Figure 2. Biome-averaged uptake and production parameters used to simulate OCS in SiB4.
Data Access
These data are available through the Oak Ridge National Laboratory (ORNL) Distributed Active Archive Center (DAAC).
SiB4 Modeled 0.5-degree Carbonyl Sulfide Vegetation and Soil Fluxes, 2000-2020
Contact for Data Center Access Information:
- E-mail: uso@daac.ornl.gov
- Telephone: +1 (865) 241-3952
References
Berry, J., A. Wolf, J.E. Campbell, I. Baker, N. Blake, D. Blake, A.S. Denning, S.R. Kawa, S.A. Montzka, U. Seibt, K. Stimler, D. Yakir, and Z. Zhu. 2013. A coupled model of the global cycles of carbonyl sulfide and CO2: A possible new window on the carbon cycle. Journal of Geophysical Research: Biogeosciences 118:842–852. https://doi.org/10.1002/jgrg.20068
Deepagoda, T.K. K.C., P. Moldrup, P. Schjønning, L.W. de Jonge, K. Kawamoto, and T. Komatsu. 2011. Density-corrected models for gas diffusivity and air permeability in unsaturated soil. Vadose Zone Journal 10:226–238. https://doi.org/10.2136/vzj2009.0137
Elliott, S., E. Lu, and F.S. Rowland. 1989. Rates and mechanisms for the hydrolysis of carbonyl sulfide in natural waters. Environmental Science & Technology 23:458–461. https://doi.org/10.1021/es00181a011
Haynes, K. D., I.T. Baker, and A.S. Denning. 2020. The Simple Biosphere Model Version 4.2: SiB4 Technical Description. https://hdl.handle.net/10217/200691
Haynes, K.D., I.T. Baker, and A.S. Denning. 2021. SiB4 Modeled Global 0.5-Degree Monthly Carbon Fluxes and Pools, 2000-2018. ORNL DAAC, Oak Ridge, Tennessee, USA. https://doi.org/10.3334/ORNLDAAC/1848
Haynes, K.D., I.T. Baker, A.S. Denning, R. Stöckli, K. Schaefer, E.Y. Lokupitiya, and J.M. Haynes. 2019. Representing grasslands using dynamic prognostic phenology based on biological growth stages: 1. Implementation in the Simple Biosphere Model (SiB4). Journal of Advances in Modeling Earth Systems 11:4423–4439. https://doi.org/10.1029/2018ms001540
Hilton, T.W., M.E. Whelan, A. Zumkehr, S. Kulkarni, J.A. Berry, I.T. Baker, S.A. Montzka, C. Sweeney, B.R. Miller, and J. Elliott Campbell. 2017. Peak growing season gross uptake of carbon in North America is largest in the Midwest USA. Nature Climate Change 7:450–454. https://doi.org/10.1038/nclimate3272
Hu, L., S.A. Montzka, A. Kaushik, A.E. Andrews, C. Sweeney, J. Miller, I.T. Baker, S. Denning, E. Campbell, Y.P. Shiga, P. Tans, M.C. Siso, M. Crotwell, K. McKain, K. Thoning, B. Hall, I. Vimont, J.W. Elkins, M.E. Whelan, and P. Suntharalingam. 2021. COS-derived GPP relationships with temperature and light help explain high-latitude atmospheric CO 2 seasonal cycle amplification. Proceedings of the National Academy of Sciences 118:e2103423118. https://doi.org/10.1073/pnas.2103423118
Kooijmans, L.M. J., A. Cho, J. Ma, A. Kaushik, K.D. Haynes, I. Baker, I.T. Luijkx, M. Groenink, W. Peters, J.B. Miller, J.A. Berry, J. Ogée, L.K. Meredith, W. Sun, K.-M. Kohonen, T. Vesala, I. Mammarella, H. Chen, F.M. Spielmann, G. Wohlfahrt, M. Berkelhammer, M.E. Whelan, K. Maseyk, U. Seibt, R. Commane, R. Wehr, and M. Krol. 2021. Evaluation of carbonyl sulfide biosphere exchange in the Simple Biosphere Model (SiB4). Biogeosciences 18:6547–6565. https://doi.org/10.5194/bg-18-6547-2021
Lawrence, D.M., and A.G. Slater. 2007. Incorporating organic soil into a global climate model. Climate Dynamics 30:145–160. https://doi.org/10.1007/s00382-007-0278-1
Meredith, L.K., K. Boye, C. Youngerman, M. Whelan, J. Ogée, J. Sauze, and L. Wingate. 2018. Coupled biological and abiotic mechanisms driving carbonyl sulfide production in soils. Soil Systems 2:37. https://doi.org/10.3390/soilsystems2030037
Meredith, L.K., J. Ogée, K. Boye, E. Singer, L. Wingate, C. von Sperber, A. Sengupta, M. Whelan, E. Pang, M. Keiluweit, N. Brüggemann, J.A. Berry, and P.V. Welander. 2018. Soil exchange rates of COS and CO18O differ with the diversity of microbial communities and their carbonic anhydrase enzymes. The ISME Journal 13:290–300. https://doi.org/10.1038/s41396-018-0270-2
Millington, R.J., and J.P. Quirk. 1961. Permeability of porous solids. Transactions of the Faraday Society 57:1200-1207. https://doi.org/10.1039/TF9615701200
Montzka, S.A., P. Calvert, B.D. Hall, J.W. Elkins, T.J. Conway, P.P. Tans, and C. Sweeney. 2007. On the global distribution, seasonality, and budget of atmospheric carbonyl sulfide (COS) and some similarities to CO2. Journal of Geophysical Research: Atmospheres 112:D09302. https://doi.org/10.1029/2006jd007665
Ogée, J., J. Sauze, J. Kesselmeier, B. Genty, H. Van Diest, T. Launois, and L. Wingate. 2016. A new mechanistic framework to predict OCS fluxes from soils. Biogeosciences 13:2221–2240. https://doi.org/10.5194/bg-13-2221-2016
Seibt, U., J. Kesselmeier, L. Sandoval-Soto, U. Kuhn, and J.A. Berry. 2010. A kinetic analysis of leaf uptake of COS and its relation to transpiration, photosynthesis and carbon isotope fractionation. Biogeosciences 7:333–341. https://doi.org/10.5194/bg-7-333-2010
Stimler, K., J.A. Berry, and D. Yakir. 2011. Effects of carbonyl sulfide and carbonic anhydrase on stomatal conductance. Plant Physiology 158:524–530. https://doi.org/10.1104/pp.111.185926
Wehr, R., R. Commane, J.W. Munger, J.B. McManus, D.D. Nelson, M.S. Zahniser, S.R. Saleska, and S.C. Wofsy. 2017. Dynamics of canopy stomatal conductance, transpiration, and evaporation in a temperate deciduous forest, validated by carbonyl sulfide uptake. Biogeosciences 14:389–401. https://doi.org/10.5194/bg-14-389-2017
Whelan, M.E., S.T. Lennartz, T.E. Gimeno, R. Wehr, G. Wohlfahrt, Y. Wang, L.M. J. Kooijmans, T.W. Hilton, S. Belviso, P. Peylin, R. Commane, W. Sun, H. Chen, L. Kuai, I. Mammarella, K. Maseyk, M. Berkelhammer, K.-F. Li, D. Yakir, A. Zumkehr, Y. Katayama, J. Ogée, F.M. Spielmann, F. Kitz, B. Rastogi, J. Kesselmeier, J. Marshall, K.-M. Erkkilä, L. Wingate, L.K. Meredith, W. He, R. Bunk, T. Launois, T. Vesala, J.A. Schmidt, C.G. Fichot, U. Seibt, S. Saleska, E.S. Saltzman, S.A. Montzka, J.A. Berry, and J.E. Campbell. 2018. Reviews and syntheses: Carbonyl sulfide as a multi-scale tracer for carbon and water cycles. Biogeosciences 15:3625–3657. https://doi.org/10.5194/bg-15-3625-2018